For several decades, fusion has been touted as the paragon of energy. It would provide an essentially unbounded supply of secure, carbon-free power and heat for non-electricity applications, while the lack of long-lived waste and the impossibility of a runaway fusion reaction only add to its value proposition. But though fusion technology has advanced radically since the first lab-based demonstration in 1932, a reaction that produces more energy than it consumes—known as a net energy gain reaction—has remained out of reach.
That could change soon. Over the past several years, fusion has leapt out of the academic domain and into the commercial, with fusion companies pushing towards net energy production, attracting nearly $5 billion in private capital, and prompting the US government to map out a “bold decadal vision” for fusion energy. With more than thirty private fusion businesses making strides towards commercial deployment on a timeline consistent with the demands of the energy transition, the time to get familiar with the industry’s internal variations, multi-sector potential, and policy needs is now.
The fusion technology landscape
Fusion involves the nuclei of two light elements overcoming the forces between them under extreme temperatures and pressures and joining, creating the nucleus of a new, heavier atom and releasing massive amounts of energy in the process. It is the phenomenon that powers the stars. In pursuit of the same self-sustaining energy production, fusion organizations are pursuing exceptionally diverse methods to get there.
The most common approach is magnetic confinement fusion, implemented by several private sector organizations along with major international fusion projects like ITER and the Joint European Torus. In fusion, the fuel takes the form of a superheated plasma, too hot to be in direct contact with any materials. Magnetic confinement fusion solves this problem by suspending the electrically conductive plasma in magnetic fields and steering it around a vacuum chamber. In 2021, Commonwealth Fusion Systems successfully demonstrated its high-temperature superconductor (HTS) magnets, setting the stage for magnetic confinement fusion to be conducted in facilities close in size to an average coal- or gas-fired power plant, rather than the gargantuan compounds in which experiments had previously been run.
The other major approach is inertial confinement fusion. This method involves heating a very small fuel “pellet” using a laser or particle beam to compress the pellet so intensely that a fusion reaction occurs, creating a small explosion and generating energy. Inertial confinement fusion follows the same principles as the fusion reactions that take place when thermonuclear weapons are detonated, but on a much smaller, nondestructive scale. In an inertial confinement fusion power plant, a steady series of these explosions would produce consistent energy output.
The biggest names in the inertial confinement space are state-run research facilities including the US-based National Ignition Facility (NIF) and the French Laser Mégajoule, but those facilities have historically been focused on nuclear weapons research. NIF, however, achieved a major milestone last year by reaching a “burning plasma regime,” in which the fusion reactions generate most of the heat in the system rather than the lasers themselves. Companies like Marvel Fusion, Focused Energy, and Xcimer Energy are vying to build off this progress and bring inertial confinement fusion to market as a reliable energy source.
Most other approaches draw from one or both of these core methods but incorporate key innovations. First Light Fusion, for example, also uses a fuel pellet, but instead of reaching critical temperatures using lasers, it fires a projectile at the pellet at an incredible velocity—with a goal of 20 kilometers per second—causing the pellet to implode and undergo fusion. Helion Energy injects and accelerates two plasmas in field-reversed configurations towards each other until they collide and fusion conditions are reached, with the aim of directly capturing the subsequent energy rather than using the heat to drive turbines. General Fusion has opted for magnetized target fusion, which uses a magnetic field to confine and compress a plasma to extreme densities but surrounds it with a liquid metal liner. HB11 Energy’s non-thermal laser-driven fusion process, supported by experimental results, would avoid the need for high temperatures entirely, while Avalanche Energy’s unique “orbitron” configuration would theoretically allow its system to fit in a backpack.
Raw materials and supply chains
In the sun, fusion involves two protons (the nuclei of hydrogen atoms) that go through a complex series of reactions called the proton-proton chain, producing energy, a helium nucleus, and various other subatomic particles along the way. For a number of reasons, though, recreating solar fusion would be infeasible on Earth, so scientists have widened their scope, pursuing fusion with other raw materials.
Most projects rely on the fusion of a deuterium and a tritium atom—isotopes of hydrogen with one and two neutrons, respectively—due to its relative ease of execution and its high experimental energy yields. Others make use of proton-boron fusion, while still others would fuse deuterium and helium-3, an isotope of helium with only one neutron instead of the usual two.
And as with all other energy sources, differences in input materials lead to differences in supply chain considerations. Deuterium makes up just under one in 5,000 hydrogen atoms in the ocean, offering a virtually inexhaustible supply. Tritium is in more limited reserve, with only 25 kilograms stockpiled globally and decaying quickly. There are paltry helium-3 resources on Earth, and attempts to mine the Moon’s natural helium-3 deposits carry doubts. Meanwhile, boron production, though set to surge considerably due to boron’s use in several clean energy applications, is currently highly concentrated, with almost two-thirds of global boron output coming from just four mines in Turkey.
But innovative solutions in varying stages of development could fill in these supply chain gaps. Deuterium-tritium (D-T) power plants are exploring the use of “breeding blankets” that contain lithium and are designed to react with the free neutrons generated by the fusion reaction, producing tritium to sustain the reaction without refueling. Some breeding blankets under development may use enriched lithium to increase reactivity, employing novel methods such as crown-ether enrichment and the ICOMAX process. Various research institutions, including the Massachusetts Institute of Technology and Oak Ridge National Laboratory, are running experiments to assess the ability of low- or no-enrichment lithium blankets to breed an adequate supply of tritium, with results expected in 2023.
To meet helium-3 supply needs, one fusion company, Helion Energy, has ventured to keep its helium-3 production planet-side, fusing deuterium atoms in a proprietary process. Those sourcing boron for fusion purposes are looking to piggyback on broader energy sector demand, with emphasis on variety, redundancy, and technological self-sufficiency.
Regulatory frameworks and approaches
Fusion is not explicitly mentioned in the Atomic Energy Act of 1954, which governs all civilian use of nuclear power and radioactive materials. The act defines atomic energy as “all forms of energy released in the course of nuclear fission or nuclear transformation.” But in 2009, upon the recommendation of its staff, the Nuclear Regulatory Commission (NRC) extended its jurisdiction to commercial fusion energy devices.
In the same decision, though, the NRC kicked the regulatory can down the road, choosing instead to wait for fusion technologies to become more mature before settling on a specific approach, or until it was compelled to by Congress. That time came in 2019, when the Nuclear Energy Innovation and Modernization Act became law and mandated the development of a technology-inclusive regulatory framework for “advanced nuclear reactors”—a category in which the statute included fusion—by the end of 2027.
To that end, the NRC has a few options, which it laid out in a recent white paper. One of them is to regulate fusion energy devices under Parts 30 through 37 of Title 10 of the Code of Federal Regulations. These provisions govern the use of “byproduct material,” which is defined in the code as all radioactive material that is not itself fissile nor fertile (meaning material that can absorb neutrons and decay into fissile material) but is instead created from fission reactions. Given the predominance of tritium in fusion reactions, the NRC has thus far regulated fusion research and development (R&D) projects under the Part 30 regulatory framework. The byproduct material framework has allowed early-stage fusion endeavors to move from milestone to milestone without overly onerous regulation. However, the approach focuses on the material used or produced within a fusion device and whether that fusion device would be considered an accelerator, so there may be some disconnects under this framework between existing regulations and the commercial-scale facilities that emerge. Limited updates or extensions to this framework, which NRC staff have explicitly indicated as their preferred route and would mirror policies currently being rolled into legislation in the United Kingdom, could make it more fit for purpose.
Another approach would be to classify fusion energy devices as “utilization facilities,” which, under the Atomic Energy Act, are facilities that use atomic energy in a way that has a bearing on national security or public wellbeing. Under NRC regulations, a utilization facility is primarily defined to be a fission reactor. Still, as the NRC noted in its 2009 paper, the NRC could expand the definition set forth in its regulations to include fusion within its utilization regulations if the NRC determined that “such devices are of significance to the common defense and security, or could affect the health and safety of the public.” But doing so would subject fusion projects to the same highly stringent set of regulations as fission reactors, which present a completely different risk profile. And it would impose additional requirements for capitalization, licensing and permitting, and workforce development.
The third regulatory option would be to create a hybrid framework, melding aspects of both byproduct material and utilization facility statutes. This would require the identification of decision criteria that could sufficiently separate different fusion designs in order to regulate each of them properly. After assessing designs based on these criteria, the NRC would then move them individually into the byproduct material or utilization facility categories. While this would ostensibly offer a level of tailored control over the regulation of commercial fusion facilities, it could also foment uncertainty among developers and investors, who would be in the dark about the requirements their project must meet until well after significant capital expenditure.
The NRC now has a little over five years to chart a regulatory course, existing or bespoke, that addresses fusion’s safety concerns yet allows the industry to grow at the necessary pace to play a sizable role in decarbonization efforts by 2050. Hearings in the next few months and an expected decision date in 2023 could move that timeline even closer.
Opportunities for policy intervention
In both allies like the United Kingdom and rivals like China, policymakers and planners are putting the pieces in place to enable commercial fusion development. For the United States to keep up, US policymakers will need to play their role in the continued growth of the industry.
One way they can do so is through public-private fusion partnership models, like a newly announced Department of Energy initiative; milestone-based, technology-neutral approaches would drive the entire industry towards maturity without bailing out companies that fail to hit targets. As fusion’s market size and companies’ investment needs grow, these programs will need to scale accordingly.
Consistent support for US national labs, universities, and government-led fusion R&D, with an express directive to assist commercialization efforts, is another key lever for policymakers. This includes ITER, NIF, the Princeton Plasma Physics Laboratory, and Advanced Research Projects Agency-Energy (ARPA-E) projects. Strong levels of annual funding for commercially-oriented projects would enable these institutions to develop a skilled workforce and find solutions to the science and materials problems that commercial fusion companies still face on their way to a net energy gain reaction.
This support should be meted out with the understanding that a net energy gain fusion reaction is not the same as a net energy gain fusion power plant. A fusion power plant will likely need colossal amounts of energy for its magnets, lasers, and other equipment, and heat-to-electricity transfer always entails some energy loss. To that end, smart public support for fusion energy would impel progress towards a deployable net energy gain fusion reaction, but it would also fund R&D for less energy-intensive facilities and more efficient capture of electricity in parallel.
Federal procurement support and coordination with international partners would help secure availability and production capacity for both primary inputs and highly complex end products like HTS magnets and lasers. And legislation strengthening and formally extending the NRC’s statutory authority could eliminate regulatory ambiguity if unforeseen issues arise during the rulemaking process.
Fusion energy could eventually underpin the entire US energy system, but it cannot do so without solid policy backing and regulatory clarity. The private capital-fueled fusion boom and international policy headway proves that the time to engender that support is now. If not, fusion ventures that prove technological maturity and reach net energy gain will run out of runway before they can turn deployable fusion energy from a quixotic idea into a staple on the grid.
Ameya Hadap is a program assistant at the Atlantic Council Global Energy Center.
Meet the author
Related content
Learn more about the Global Energy Center
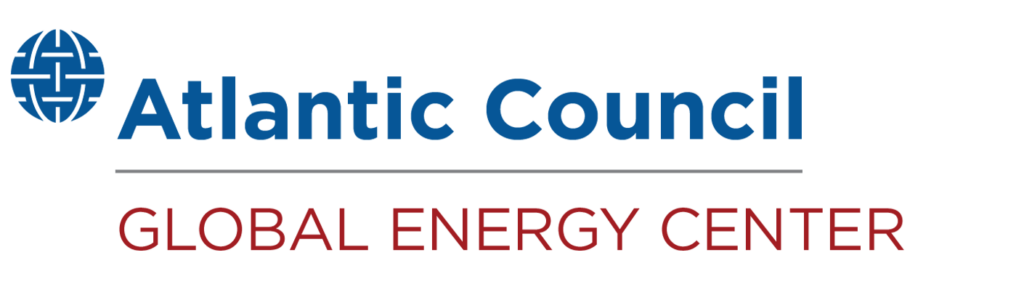
The Global Energy Center develops and promotes pragmatic and nonpartisan policy solutions designed to advance global energy security, enhance economic opportunity, and accelerate pathways to net-zero emissions.
Image: A diagram of an atom. (Sergey Nivens, Adobe Stock, Standard License) https://stock.adobe.com/license-terms#standardLicenses